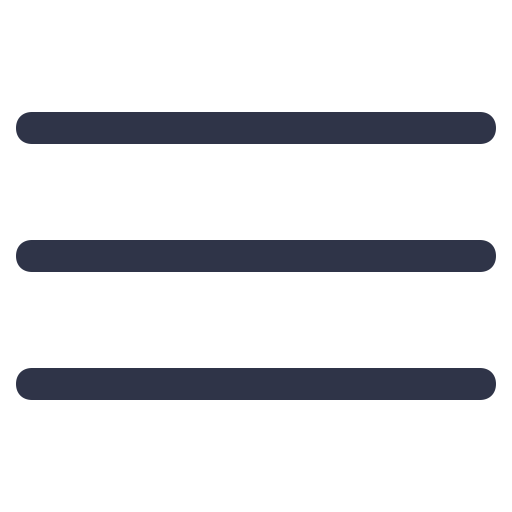
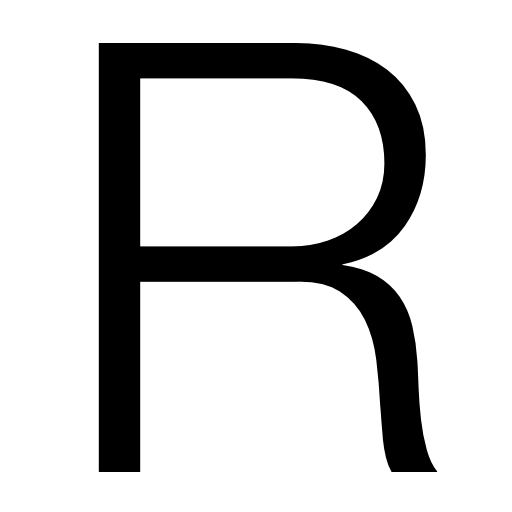
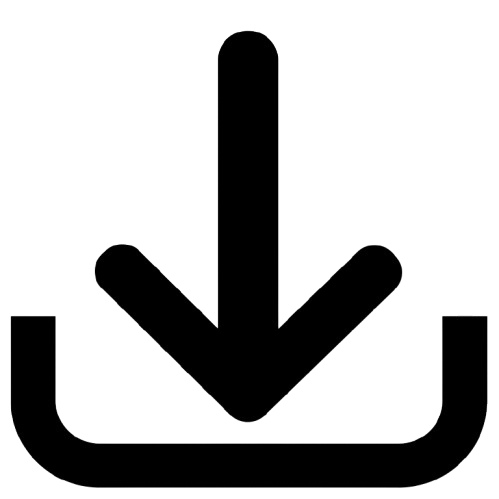
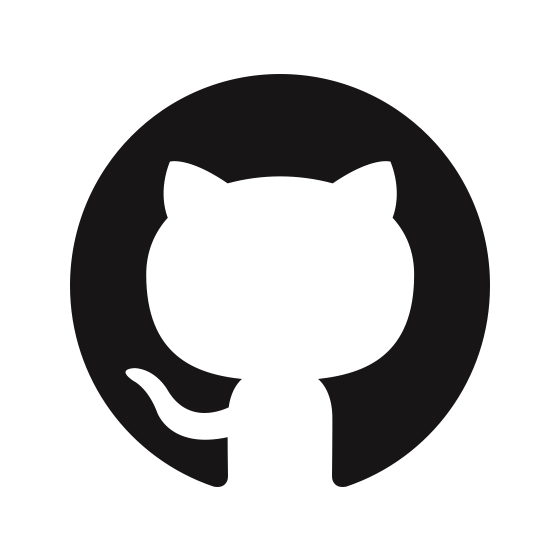
6.2 Detecting particles
6.2.1 Particle interactions with matter
In Part I, we discussed the interpretation of fundamental particles as irreps of the Poincaré group and quantum excitations of fields. In experimental physics, we have yet another interpretation: “a particle is an object that interacts with your detector such that you can follow its track” (W. Riegler [176]).
Which is to say, in order to detect particles, they must interact with the detector material and transfer energy in a way that we can measure. Out of the myriad particles produced in LHC proton-proton collisions, the are only eight particles stable enough to reach the CMS detector and be detected are listed in Table 6.1. Neutrinos are also stable, but are too weakly interacting to measure with the CMS detector, which means it is vital to measure the energy of all the other particles hermetically; the presence of neutrinos can then be inferred by energy conservation, or “missing energy” carried by neutrinos.
Charged particles
Out of these eight, the charged particles can interact electromagnetically with matter through:
- Ionization and excitation of atoms: inelastic scattering with atomic electrons and elastic scattering from nuclei, respectively. The average energy loss per distance of a particle due to ionization is given by the famous Bethe-Bloch formula [177].
- Bremsstrahlung: photon radiation because of (de-)acceleration in the electric field of nuclei;
- Cherenkov effect: photon radiation due to the particle moving faster than the speed of light in the medium;
- and transition radiation: photon radiation due to the crossing a boundary between two different dielectrics.
Generally, for “heavy” charged particles (of mass electron mass), electromagnetic interactions are dominated by ionization and excitation, while for electrons and positrons, Bremsstrahlung is dominant at higher energies.
The presence and angle of Cherenkov radiation depends on the particle momenta, a fact which is often exploited for “particle identification” (PID) by distinguishing particles of different masses at given momenta. For example, LHCb uses ring-imaging Cherenkov (RICH) detectors to distinguish hadrons, which is critical for -physics [178]. CMS, on the other hand, did not prioritize PID and cannot accurately distinguish between the different hadrons beyond their charge.
Photons
Photons primarily interact through:
- The photoelectric effect: absorption by an atom causing the ejection of an electron, dominant at low energies, ;
- Compton scattering: incoherent scattering off an atomic electron, dominant at intermediate energies, ;
- and pair production: converting into electron-positron pairs in the Coulomb field of nuclei, dominant at high energies, .
The combination of these effects means that as high energy electrons and photons propagate through the detector material, they produce a cascade of secondary particles, called an electromagnetic shower, which are analyzed to infer the presence and overall energy of the originating particle.
It is often convenient to characterize detector materials by their radiation length (), which is the mean distance into the material over which high-energy electrons lose of their energy due to Bremsstrahlung, and of the mean free path of photons before pair production.
Hadrons
Finally, high energy hadrons can also interact with atomic nuclei through the strong force, losing energy through further particle emissions which create their own hadronic shower. As a large fraction of these emitted particles are neutral pions, which decay immediately into photons, hadronic showers are also often accompanied by electromagnetic sub-showers. We can characterize materials for hadron detection similarly by their nuclear interaction length (), the mean free path between nuclear interactions.
6.2.2 Types of detectors
Tracking detectors
The earliest particle detectors were gaseous ionization chambers. Charged particles passing through these detectors ionize the gas along their trajectory, creating visible tracks, which can be captured, for example, by using an electric field to push the ions towards photographic film. The most notable examples are cloud chambers, which were prominent in the first half of the 20th century, and led to the discovery of the positron, muon, and kaon via cosmic rays. The Nobel Prize was awarded to Charles Wilson in 1927 and Carl Anderson in 1936 for the invention and development of the cloud chamber, respectively.
Tracking detectors have since continuously evolved, such as through the use of liquid media in bubble chambers and charged wires to produce electric fields and read out ionization signals electronically in wire chambers, both of which again led to Nobel Prizes for their inventors, Donald Glaser and Georges Charpak, respectively. The Gargamelle bubble chamber at CERN notably led to the discovery of weak neutral currents [144] (see Chapter 4.2.1).
More recently, a significant advancement in tracking detectors has been achieved through the use of semiconductors such as silicon. A - semiconductor diode [179, 180] effectively forms an ionization chamber as well, where charged particles passing through will create electron-hole pairs whose charge can be collected and recorded. Semiconductor detectors can have lower ionization energies, higher granularity, better position and time resolution, and strong radiation tolerance, while also being able to leverage innovations and state-of-the-art fabrication techniques from the semiconductor industry.
Thus, there has been a gradual shift towards their use, particularly in collider physics. Here, tracking detectors are crucial for (1) vertexing — measuring particle tracks precisely to determine the point of collision, or the “vertex” — and (2) measuring the curvature of charged-particle trajectories in a magnetic field to determine their momenta. Silicon trackers, for example, were employed for vertexing in all LEP experiments and the CDF and DØexperiments at the Tevatron. The CMS detector is notably the first to use silicon for its entire tracking volume.
Semiconductor trackers are, however, more expensive per unit area. Hence, gaseous and liquid detectors remain prevalent in particle physics, particularly where large volumes are required, such as in neutrino experiments and the CMS muon system.
Calorimeters
Calorimeters are detectors designed primarily to measure the energy of particles. They can be either homogeneous, where the entire volume of the detector can both absorb and measure the energy of the shower; or, sampling, where separate “passive” layers which absorb energy and initiate the shower are interleaved with “active” layers to measure the energy. Sampling calorimeters are less precise than homogeneous calorimeters, but are more cost-effective, especially when large volumes are required. CMS employs both types of calorimeters, and primarily uses scintillation — photon emission due to atomic (de-)excitation from charged particles — to capture and measure energy.
Generally in collider physics trackers are designed to have short radiation lengths, to minimize particle energy loss, and calorimeters as long a radiation length as possible, to capture the entire energy of electromagnetic or hadronic showers. In addition to their radiation and nuclear interaction lengths, calorimeter materials are characterized as well by their Molière radius (), which is the radius of a cylinder containing, on average, 90% of an incident electron or photon’s electromagnetic shower energy. It is approximately related to as:
| (6.2.1) |
where is the atomic number of the material.
General-purpose detectors
Designing a general-purpose detector, such as CMS, requires a careful optimization of several factors, including high efficiency and resolution for as many of the particles in Table 6.1, radiation hardness, cost effectiveness, and more. A typical compromise in particle physics has been a “layered” detector design, as shown in Figure 6.3, with a thin (in radiation and nuclear interaction lengths) innermost tracker for precise vertexing and momentum measurements, followed by thick calorimeters to measure particle energies, and finally dedicated detectors to identify and measure high energy muons that are able to penetrate the previous layers. The CMS detector follows this general philosophy, as shown in Figure 6.4.